The Universe Is Always Looking
The one thing you probably understand about quantum physics is actually a poor metaphor for the modern state of the field.
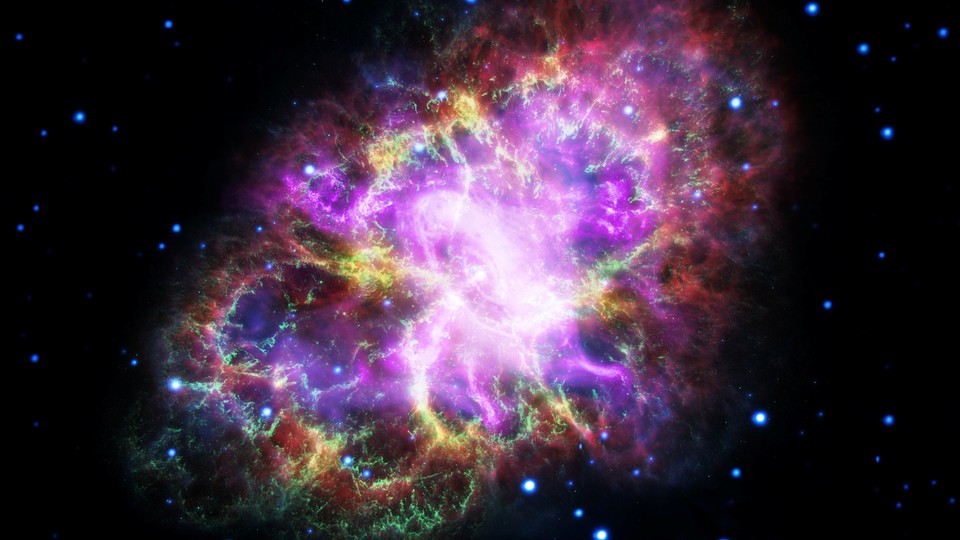
I have suggested before that the one thing “everyone knows” about quantum mechanics is that the quantum world is fuzzy and uncertain. Actually, there’s another thing, too. “Everyone” has heard of Schrödinger’s cat.
That’s why there are so many jokes about it. Schrödinger is driving along when he is pulled over by a policeman. The officer looks the car over and asks Schrödinger if he has anything in the boot.
“A cat,” Schrödinger replies.
The policeman opens the boot and yells, “Hey! This cat is dead!”
Schrödinger replies angrily, “Well, he is now.”
As physics jokes go, it’s not so bad. At the least, it illustrates what a good job Schrödinger did in finding an image catchy enough to become a cultural meme.
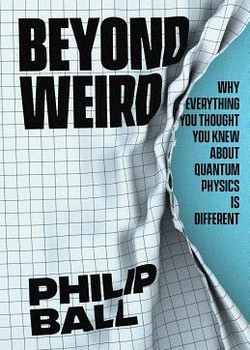
One might even say that he was too successful. The cat is still hauled out today as if to imply that we’re as puzzled as ever by the mere fact that the quantum world at small scales turns into the world of classical physics at human scales. The fact is, however, that this so-called quantum-classical transition is now largely understood. Things have moved on, and we can state much more precisely than Schrödinger and his contemporaries could why and how quantum becomes classical. The answer is both elegant and rather astonishing.
For quantum physics is not replaced by another sort of physics at large scales. It actually gives rise to classical physics. Our everyday, commonsense reality is, in this view, simply what quantum mechanics looks like when you’re six feet tall. You might say that it is quantum all the way up.
The question, then, is not why the quantum world is “weird,” but why ours doesn’t look like that, too.
In Schrödinger’s day, traversing the quantum-classical transition seemed like crossing an ocean between two continents: Drawing a border somewhere in the open sea might be an arbitrary exercise, but the continents are undeniably distinct. The land of the quantum, Schrödinger said, is random and unpredictable, yet the classical realm is orderly and deterministic because it depends only on statistical regularities among that atomic-scale chaos.
Schrödinger dreamed up his “diabolical” (his word) thought experiment in 1935. It was intended as a challenge to Niels Bohr’s interpretation of quantum mechanics, toward which Schrödinger shared a great deal of Albert Einstein’s skepticism.
It was all very well for Bohr to impose a strict separation of quantum and classical, and to make observation the process by which they are distinguished—but what, then, if the quantum and the macroscopic are coupled without any observation taking place? Schrödinger was looking for what he called a “ridiculous case”: a reductio ad absurdum, not to be taken literally, in which we are confronted by a superposition of macroscopic states that seems not just bizarre (such as a large object being in “two places at once”) but logically incompatible. Einstein raised the prospect of a keg of gunpowder being in a superposition of exploded and unexploded states, and Schrödinger upped the ante with his cat, whose life or death is yoked to a quantum event such as the radioactive decay of an atom. If, as Bohr said, the state of the atom is undetermined (in a superposition) until we look, then so must be the state of the cat.
Schrödinger’s cat forces us to rethink the question of what distinguishes quantum from classical behavior. Why should we accept Bohr’s insistence that they’re fundamentally different things unless we can specify what that difference is?
We might then be inclined to point to features that classical objects like coffee cups have but that quantum objects don’t necessarily have: well-defined positions and velocities, say, or characteristics that are localized on the object itself and not spread out mysteriously through space. Or we might say that the classical world is defined by certainties while the quantum world is (until a classical measurement impinges on it) no more than a tapestry of probabilities, with individual measurement outcomes determined by chance. At the root of the distinction, though, lies the fact that quantum objects have a wave nature—which is to say, the equation Schrödinger devised in 1924 to quantify their behavior tells us that they should be described as if they were waves, albeit waves of a peculiar, abstract sort that are indicative only of probabilities.
It is this waviness that gives rise to distinctly quantum phenomena like interference, superposition, and entanglement. These behaviors become possible when there is a well-defined relationship between the quantum “waves”: in effect, when they are in step. This coordination is called “coherence.”
The concept comes from the science of ordinary waves. Here, too, orderly wave interference (like that from double slits) happens only if there’s coherence in the oscillations of the interfering waves. If there is not, there can be no systematic coincidence of peaks and troughs and no regular interference pattern, but just random, featureless variations in the resulting wave amplitude.
Likewise, if the quantum wave functions of two states are not coherent, they cannot interfere, nor can they maintain a superposition. A loss of coherence (decoherence) therefore destroys these fundamentally quantum properties, and the states behave more like distinct classical systems. Macroscopic, classical objects don’t display quantum interference or exist in superpositions of states because their wave functions are not coherent.
Notice how I phrased that. It remains meaningful to think of these objects as having wave functions. They are, after all, made of quantum objects and so can be expressed as a combination of the corresponding wave functions. It’s just that the wave functions of distinct states of macroscopic objects, such as a coffee cup being in this place and that place, are not coherent. Quantum coherence is essentially what permits “quantumness.”
There is no reason (that we yet know of) why, in principle, objects cannot remain in coherent quantum states no matter how big they are—provided that no measurement is made on them. But it seems that measurement somehow does destroy quantum coherence, forcing us to speak of the wave function as having “collapsed.” If we can understand how measurement unravels coherence, then we would be able to bring measurement itself within the scope of quantum theory, rather than making it a boundary where the theory stops.
The crucial factor in understanding quantum decoherence is that ubiquitous entity present but largely ignored in all scientific studies: the surrounding environment. Every real system in the universe sits somewhere, surrounded by other stuff and interacting with it. Schrödinger’s cat might be placed inside a sealed box, but there must be air in there for the cat to have any chance of staying alive. And the cat is resting on a surface of some kind, exchanging heat with it.
In quantum mechanics, the environment has a central role in how things happen. It turns out to be precisely what conjures the illusion of classical physics out of the quantum soup.
It’s often suggested that quantum states such as superpositions are delicate and fragile. Put them in a noisy environment (the story goes), and all that jiggling and shaking by the surroundings destroys these frail quantum states, collapsing wave functions and shattering superpositions. But this isn’t quite right. Indeed, why should quantum states be fragile if quantum mechanics supplies the most fundamental description of the universe? What kinds of laws are these, if they give up the ghost so easily?
The truth is that they don’t. Quantum superpositions of states aren’t fragile. On the contrary, they are highly contagious and apt to spread out rapidly. And that is what seems to destroy them.
If a quantum system in a superposed state interacts with another particle, the two become linked into a composite superposition. That is exactly what quantum entanglement is: a superposed state of two particles, whose interaction has turned them into a single quantum entity. It’s no different for a quantum particle off which, say, a photon of light bounces: The photon and the particle may then become entangled. Likewise, if the particle bumps into an air molecule, the interaction places the two entities in an entangled state. This is, in fact, the only thing that can happen in such an interaction, according to quantum mechanics. You might say that, as a result, the quantumness—the coherence—spreads a little further.
In theory, there is no end to this process. That entangled air molecule hits another, and the second molecule gets captured in an entangled state, too. As time passes, the initial quantum system becomes more and more entangled with its environment. In effect, we then no longer have a well-defined quantum system embedded in an environment. Rather, system and environment have merged into a single superposition.
Quantum superpositions are not, then, really destroyed by the environment, but on the contrary infect the environment with their quantumness, turning the whole world steadily into one big quantum state.
This spreading is the very thing that destroys the manifestation of a superposition in the original quantum system. Because the superposition is now a shared property of the system and its environment, we can no longer “see” the superposition just by looking at the little part of it. We can’t see the wood for the trees. What we understand to be decoherence is not actually a loss of superposition but a loss of our ability to detect it in the original system.
Only by looking closely at the states of all these entangled particles in the system and its surroundings can we deduce that they’re in a coherent superposition. And how can we hope to do that—to monitor every reflected photon, every colliding air molecule? No—once the quantumness has leaked out into the environment, in general we’ll never be able to concentrate the superposition back on to the original system.
Decoherence, then, is a gradual and real physical event that occurs at a particular rate. For some relatively simple systems, we can use quantum mechanics to calculate that rate: to work out how long it takes for decoherence to sabotage the possibility of observing, let’s say, interference between the wave functions of a quantum object in two different positions. The farther apart in space those two positions are, the faster the coherence between them will become entangled with, and leak away into, the environment.
Take a microscopic dust grain floating in the air of my study, a hundredth of a millimeter across. How quickly do two positions of the grain decohere if their separation is (say) about the same size as the width of the grain itself, so that they don’t overlap? Ignore photons for now—let’s say the room is dark—and just think about the interactions between the grain and all the air molecules around it. Quantum calculations show that decoherence then takes about 10-31 seconds.
That’s so short that we can almost say that decoherence is instantaneous. It happens in less than a millionth of the time it takes for a photon, traveling at the speed of light, to pass from one side of a single proton to the other. So if you think you’re going to see a quantum superposition of nonoverlapping position states of a dust grain in my study, think again.
For microscopic objects, we really can avoid decoherence. That’s the whole point—it’s why we really can do experiments on atoms, subatomic particles, and photons that reveal them to be in quantum superpositions. For a large molecule (the size of a protein, say), decoherence happens within 10-19 seconds if it were floating in the air around us—but in a perfect vacuum at the same temperature, it could stay coherent for more than a week.
Decoherence is what destroys the possibility of observing macroscopic superpositions—including Schrödinger’s live/dead cat. And this has nothing to do with observation in the normal sense: We don’t need a conscious mind to “look” in order to “collapse the wave function.” All we need is for the environment to disperse the quantum coherence. We obtain classical uniqueness from quantum multiplicity when decoherence has taken its toll.
Einstein once expressed his exasperation at Bohr’s position on quantum weirdness to the young physicist Abraham Pais. “I recall,” Pais wrote, “that during one walk Einstein suddenly stopped, turned to me and asked whether I really believed that the moon exists only when I look at it.” With an understanding of decoherence, we have an answer to Einstein’s question. Yes, it is there when no one observes it—because the environment is already, and without cease, “measuring” it. All of the photons of sunlight that bounce off the moon are agents of decoherence, and are more than adequate to fix its position in space and give it a sharp outline. The universe is always looking.
This post is adapted from Ball’s new book, Beyond Weird: Why Everything You Thought You Knew About Quantum Physics Is Different.